A key feature of malignant tumors is that they recur, locally or remotely. Those responsible for distant recurrence are believed to be “metastatic cells” detached from the primary tumor, which circulate through lymphatic or blood capillaries, and eventually deposited in a new place of the body (1). Given the inherited characteristics of the secondary tumors, even if they occur years after the growth of the first tumor, it is assumed that these metastatic cells are released by the primary tumor. This implies that these cells were occult and inactive all along the remission period, in a sort of dormant state. Dormancy, hypoxia tolerance, anoikis resistance, and immune escape, are some of the apparent qualities attributed to malignant metastatic cells, those seen as super-cells.
One of the unanswered questions in oncology is: Are the metastatic cells born with the cancerous transformation, or does this develop during the tumor progression? That query may also be phrased as: Are they a genetic effect of the initial malignant transformation or perhaps a tumor progression environment epigenetic influence?
In the second half of the last century, it was widely accepted that metastatic cells were a portion of the tumor cell population, accepting as a fact the “intrinsic heterogeneity of tumor cells” (2). However, hundreds, if not thousands of investigations have tried to find a definitive answer to those questions, an answer which is not yet available.
Nevertheless, the influence of the surroundings on the definition of the metastatic cell phenotype is of outstanding relevance today (3). The modern understanding of the heterogeneity of tumor cells, including the metastatic phenotype, contemplates a rainbow of intrinsic and extrinsic inducing factors (4), among them, local hypoxia has rightly acquired preeminent attention (5).
A universal finding in tumor tissues is the existence of regions with oxygen deficiency (hypoxia), with respect to the partial oxygen pressure of normal tissues. Hypoxia induces the expression of factors (HIFs) that act as circumstantial regulators that modulate the expression of genes related to the promotion of angiogenesis, contribute to the stress of glucose metabolism, promote the renewal of the extracellular matrix, help reprogramming of stem cells, contribute to epithelial-mesenchymal transition, support the activation of cell motility and invasiveness (promotion of metastases), etc. (6, 7). Moreover, they confer resistance to multiple drugs, increase the tolerance to ionizing radiation, and confer immune escape.
The in-vitro research on the hypoxia effect on tumor cells has a specific need: to incubate the cultures in special environments, capable of reducing the oxygen partial pressure of the culture medium (M-PO2). This is commonly achieved by isolating the cultures inside hermetic chambers in which most of the air is replaced by exogenous nitrogen (hypoxia chambers). By drastically reducing, the oxygen of the environment, the cell culture medium is forced to outwardly diffuse its dissolved oxygen, which, depending on the medium volume, provokes over 6 to 10 hours, a drop of the M-PO2 until this equals the air oxygen partial pressure (Atmosphere, A- PO2). With good instruments, this is a reliable method, because precise and fast sensors combined with precise electro-valves, and a well-designed PLC software, will maintain a relatively stable oxygen concentration inside the chamber. That stability can be maintained as long as a test or necessary maneuvers do not disrupt the hermetic enclosure (i.e. Chamber door opening).
The only restriction of this method is that only a single layer of oxygen concentration is testable per chamber or incubator, forcing the user to perform multiple cultures in a serial publication of different oxygen concentration experiments, and with the consequent inconsistency of the media conditions, and the extravagant expenditure of time and resources.
The irruption in the market of the DRC or “snorkel chambers” (Petaka G3), changed forever this experimental scenario. In these cell culture chambers the oxygen of the media is not lost in the atmosphere, rather, it is consumed by the cells living in the chamber. The reduced M-PO2 of the medium could become lower than the needs of the cell population because as long as the internal M-PO2 is higher than the external A-PO2 of the normal open atmosphere (M-PO2 > A-PO2) the inflow of external oxygen will continue. Since the live cell population will continue consuming oxygen it will reach a balance of the internal and the external oxygen tensions, (M-PO2 = A-PO2), enough to maintain the life of these specific cells of the culture (phase of sustainable consumption of oxygen). At this point, the oxygen inflow stops, maintaining a stable concentration of dissolved oxygen, but exposed to the cellular oxygen consumption changes. At this point, if the cells consume more oxygen, the internal M-PO2 and external A-PO2 become unbalanced, now in favor of the external (M-PO2 < A-PO2), allowing the inflow of oxygen, increasing the internal M-PO2. Until the balance M-PO2 = A-PO2 is reached again, and the oxygen inflow stops. This cycle is repeated again and again responding to the specific cell population metabolic activity.
In addition, these bioreactors may have an internal oxygen fluorescent sensor, at the same level of the cell monolayer (Petaka G3 DOsensor), that provides continuous readings of the dissolved oxygen concentration of the medium at the cellular level, vital information for cell biology and cancer research.
The progression of the M-PO2 reduction, imposed by the cell metabolism, provides a continuous gradient of the available dissolved oxygen, making it possible to correlate M-PO2 with other cell states, overwhelming the limitation of the fixed amount imposed with standard hypoxia chambers.
Moreover, in a DRC, the oxygen diffusion system is controlled by the cellular population’s own metabolism, if the dissolved oxygen in the medium is not consumed, no more oxygen enters, but if the cells consume it, the oxygen continues to enter the medium through diffusion, so that an oxygen concentration is reached that marks the minimum limit of cellular respiration, under which the cells minimize or suppress OX-PHOS and proceed to anaerobic glycolysis. Thus, when the available oxygen falls below a certain limit, the cells stop consuming it, then, the oxygen intake exceeds consumption and the oxygen concentration increases in the medium; Immediately, that increased concentration is perceived by the cell population, which resumes the respiration, so that the oxygen consumption returns to exceed the admission, with which soon the concentration will decrease until inhibiting the cellular respiration. And so on, creating a dynamic equilibrium, hypoxia-reoxygenation, a situation similar to ischemia and vasodilation. The average level of oxygen concentration in that balance indicates the physioxia limit of the studied cell population. In this mode, the cells can be sustained for a long time exposed to the limit of their demand for oxygen, and to see how they behave in these conditions, what is the process of adjustment, if any, to these accidental environments.
In contrast, studies with hypoxia, performed with a single concentration of oxygen, unchanged and sustained for a limited period of time, do not conform to the characteristics of the cells studied. Single point hypoxia studies are limited to forcing an oxygen concentration, so low and so sudden that any cell will suffer acute stress, or death if it is sustained long enough.
Many papers have suggested that hypoxia in the tumor environment increases the resistance of tumor cells to anoikis (8), and its malignancy, attributable to several factors, including increased endurance to adverse immune responses, chemotherapy agents and radiations.
Here it is described as an experimental example of the behavior of HeLa cells, a tumor cell line derived from a human cervical cancer, in an environment
Experiment structure:
Twenty cap-vented T25 flasks, 8 PetakaG3 LOT and 4 PetakaG3 DO-sensor were seeded with 70,000 HeLa cells/cm2 each (1,75 million cells in T25 and 5.25 million in Petaka G3). All devices contained DMEM media with 4,500 mg/l glucose, protected with penicillin-streptomycin, and supplemented with 10% FBS. All devices were maintained in CO2 incubators at pO2 = 150 mmHg and 5% CO2, for 15 days, 30 days, and 45 days without media renewal (in vitro starvation).
Cell counts on phase-contrast microscopy (cultures in a monolayer).
The cells are allowed to grow in all cultures at 37OC and are periodically counted on phase-contrast images by means of stereological technique (9), so as not to alter their growth evolution.
Measurement of dissolved oxygen in the medium of Petaka G3 DO-sensor with growing cultures. –
In these devices, the dissolved oxygen was monitored from the start of the experiment for periods of 72 hours, during which measurements were drawn with a frequency of 1 reading every 15 minutes, automatically stored in excel tables in the memory of a data processor.
Doubling time calculation
After each cell count, the doubling time of that period of cell population growth is calculated according to the equation below, where t is the time of laps, NC number of cells, NCi the initial number of cells and NCf the final number of cells within the lapse:
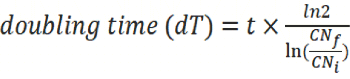
Cell viability test.
The Trypan blue exclusion test was the standard procedure to assess the percentage of viable cells in each culture.
Regeneration potential test.
The regeneration potential of each cell population, exposed to specific oxygenation conditions, was assessed with a protocol variant of the platting efficiency test, The variation involves the removal the old medium at the end of each experiment, leaving the cells untouched in the culture device, adding new media, and counting the colonies of living cells after 7 days of incubation, and assess if these colonies are able to grow up to 75% confluent in 15 more days. The evaluation of this test is binary, “able” (+) or “unable’ (-) of producing colonies, and regenerate a monolayer population. All cultures were processed according to table 1.

Results
- Measurements of dissolved oxygen in the medium of T-25 cultures. –
After each experimental period. The media of the T flasks, also incubated in the atmosphere of 21% oxygen, was collected and the DO measured, showing a uniform average concentration of 6.7 mg/l, equivalent to 150 mmHg of PO2.
- Synchronous cell growth and Oxygen partial pressure reduction in Petaka G3 DOsensor cultures (Fig. 1).
In Petaka G3 DO-Sensor cultures, dissolved oxygen was monitored from the beginning of the experiment for periods of 72 hours, during which measurements were made with a frequency of 1 reading each 15 minutes, automatically stored in Excel tables in the memory of a data processor. A whole series of measurements showed that dissolved oxygen had dropped from 150 mmHg, in the first 12 hours of cell culture development, and remained stable within an average of 19.5 mmHg, equivalent to a T flask incubated in an atmosphere of 2.73 % oxygen (Fig 1A)
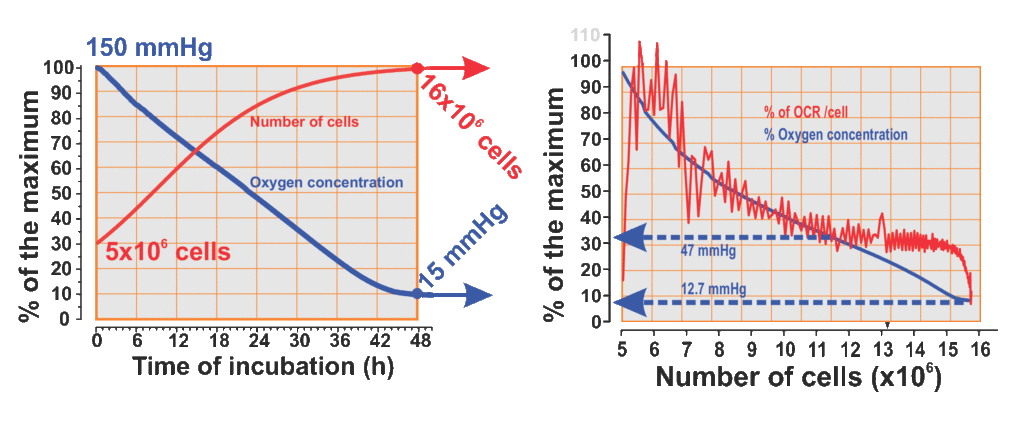
In these cassettes the cells grew normally and a whole series of measurements showed that dissolved oxygen had gone down from 150 mmHg, in the first 48 hours of cell culture development, until the cell population was close to 16 million cells, and remained stable within an average of 12.03 mmHg as long as the cell population was close to 16 million (Fig 1A). Once this moment of the culture has been reached, the remaining oxygen is calculated for each cell, with which an interesting result is obtained, in the cell microenvironment remains 1.2 pM/L of dissolved oxygen, which, according to the stability of both, the oxygen content in the medium and the cell population, suggests that it is enough to maintain a regular HeLa cell life. Which in terms of experiments inside hypoxia chambers, is equivalent to cells cultured in medium exposed to an atmosphere of the exiguous oxygen concentration of 1.1927×10-7M/L equivalent to a T25 flask incubated in an atmosphere of 1.97% oxygen. When the oxygen level stabilized, the calculation of the amount of oxygen per cell pinpoints to less than 100 fM / cell, however, with that amount these tumor cells continue living for days (Fig. 1B).
Moreover, the oxygen consumption rate (OCR) of these HeLa cells varies (Fig. 1B, red line) in response to the oxygen concentration of the medium (Fig. 1B, blue line), in well-defined sections of the hypoxia gradient. Above 60 mmHg, the oxygen consumption rate shows unusual irregularity and intensity, coinciding with the initial phase of cell population growth, when the cells are more unsynchronized. It is known that HeLa cells consume more oxygen, when they are in G0 and S phase, less oxygen in G2 phase, and extremely little oxygen during the mitosis. Between 35 mmHg and 60 mmHg the increase in cell population tends to be linear and the OCR decreases with a gentle slope and with a clear tendency to stabilize. Beneath 35 mmHg, between 25 mmHg and 36 mmHg the OCR remains in a quite stable average, and below 25 mmHg the OCR drops in a fast-parabolic fall ending in very low oxygen concentration, about 8.5 mmHg.
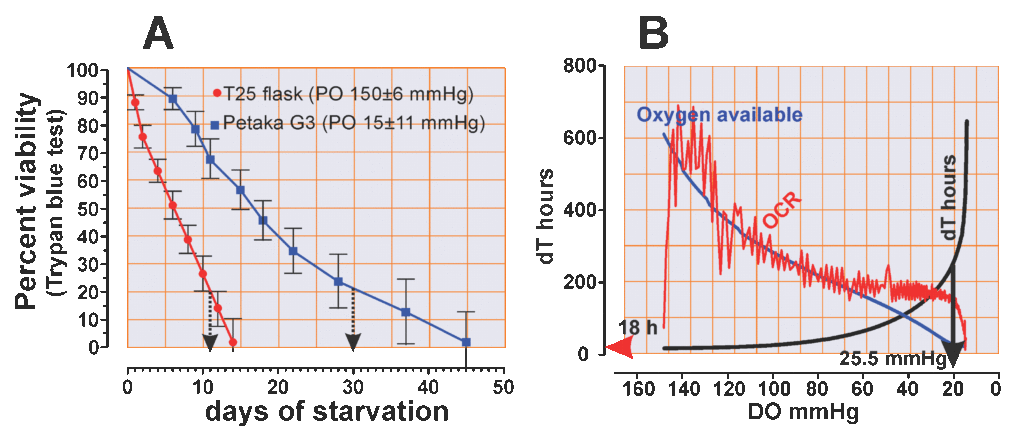
When the PO2 drops to around 20 mmHg, the doubling time of the cell population increases from 16 hours to more than 200 hours, and the more the PO2 decreases below 20 mmHg (Fig. 2B, black line), the greater the doubling time extension, which reaches 600 hours in the last section of the experiment (Fig 2B)
- Cell viability and regeneration potential under PO2 of 150 mmHg
HeLa cells under PO2 of 150 mmHg may survive for 14 days without media change; furthermore, 25% of the cells were unable to exclude the trypan blue after 10 days of starvation (Fig 2A), After 30 days of culture only 4 of 7 flasks demonstrated regeneration potential (test 57%), and after 45 days all 6 cultures were unable to develop colonies. From these 4 cultures capable of producing colonies, only 3 (75%) were able to grow up to 75% confluent monolayer (Table 1)
- Cell viability and regeneration potential under PO2 of 25 mmHg
Of the HeLa cells maintained under PO2 below 25 mmHg in the Petaka G3 cassette, 25% were viable after 30 days without media change; however, showed significant cell loss and cell stress signs, including attachment fragility (Fig. 3A). Moreover, after 30 days starvation under 25 mmHg of PO2, 100% of the cultures were able of producing colonies (Fig.3 B), and after 45 days starvation, 3 of 6 passed this test (50%) under these PO2 conditions and, from those, all 3 were able regenerating the monolayer population. Table 1, and Fig. 2B)

Interpretations
From the analysis of oxygen consumption, parallel to the growth of the cell population, it can be inferred that tumor cells, with HeLa as an example, can adjust their OCR to the environmentally available oxygen, and therefore can adjust the level of energy consumption to the oxygen available in the medium, without suspending their growth and basic physiologic functions. Nevertheless, the analysis of changes in doubling time indicates that tumor cells reduce their growth rate under hypoxia, which implies that the level of oxygen influences the molecular processes involved in the proliferation of these cells (10). This reduction of growth is extreme when the PO2 is less than 10 mmHg.
However, the regenerative potential tests show that these cells are not irreparably damaged. Rather, they remain in an apparently dormant state, with a low proliferation rate, with apparent enhanced resistance, and are capable of forming colonies that can fully regenerate the cell population when suitable oxygen and nutrient conditions are restored.
With the in-vitro assay data shown here, the possibility of tumor recurrence is verified from cells with very slow activity, that could be called “dormant cells”, after a limited period of time of days in-vitro, but in-vivo, these periods can be repeated numerous times, lengthening the apparent tumor remission period to months or years due to metastatic cells maintained in inactive proliferation or pausing. This, along with other similar experiments, allows the understanding that tumor cells can grow or simply maintain in environments with oxygen tensions below 18 mmHg. That low oxygen environment lengthens doubling time and increases the life span of tumor cells in a silent manner, immersed in oxygen adverse conditions, suspending its growth capacity until the environment oxygenation levels become greater than 40 mmHg. At which time it can attach, metabolize and begin to grow again.
REFERENCES
1.- K Pantel, K.,RH Brakenhoff, RH.,(2004). Dissecting the metastatic cascade. Nature reviews cancer, 2004 – nature.com
2.- Lawson, DA., Kessenbrock. K., Davis, RT., Pervolarakis, N., Werb, Z. (2018). Tumour heterogeneity and metastasis at single-cell resolution.). Nature Cell Biology , 20,1349–1360
3.- Andersen LMK, Wegner CS, Simonsen TG, Huang R, Gaustad JV, Hauge A, Galappathi K, Rofstad EK..(2017). Lymph node metastasis and the physicochemical micro-environment of pancreatic ductal adenocarcinoma xenografts. Oncotarget. ;8(29):48060-48074.
4.- Zhang H, Yang Q, Lian X, Jiang P, Cui J. (2019). Hypoxia-Inducible Factor-1α (HIF-1α) Promotes Hypoxia-Induced Invasion and Metastasis in Ovarian Cancer by Targeting Matrix Metallopeptidase 13 (MMP13). Med Sci Monit.;25:7202-7208:
5.- Schito, L . and Semenza, G.L. , (2016) Hypoxia-Inducible Factors: Master Regulators of Cancer Progression Trends in Cancer, Vol. 2, No. 12. 758-770.
6.- Yu Y, Min Z, Zhou Zhihang, Linhong M, Tao R, Yan L, Song H.(2019) Hypoxia-induced exosomes promote hepatocellular carcinoma proliferation and metastasis via miR-1273f transfer. Exp Cell Res. 1;385(1):111649.
7.- Kelly A. Whelan & Mauricio J. Reginato (2011) Surviving without oxygen: Hypoxia regulation of mammary morphogenesis and anoikis, Cell Cycle, 10:14, 2287-2294
8.- Baba K, Kitajima Y, Miyake S, Nakamura J, Wakiyama K, Sato H, Okuyama K, Kitagawa H, Tanaka T, Hiraki M, Yanagihara K, Noshiro H. (2017) Hypoxia-induced ANGPTL4 sustains tumor growth and anoikis resistance through different mechanisms in scirrhous gastric cancer cell lines. Sci Rep. 7(1):11127.
10- Saxena K, Jolly MK. 2019) Acute vs. Chronic vs. Cyclic Hypoxia: Their Differential Dynamics, Molecular Mechanisms, and Effects on Tumor Progression. Biomolecules.98). : E339
11.- Mas-Bargues C, Sanz-Ros J, Román-Domínguez A, Inglés M, Gimeno-Mallench L, El Alami M, Viña-Almunia J, Gambini J, Viña J, Borrás C. (2019) Relevance of Oxygen Concentration in Stem Cell Culture for Regenerative Medicine. Int J Mol Sci. 8;20(5). PII: E1195.